Translating RNA: Mechanisms and Implications in Biology
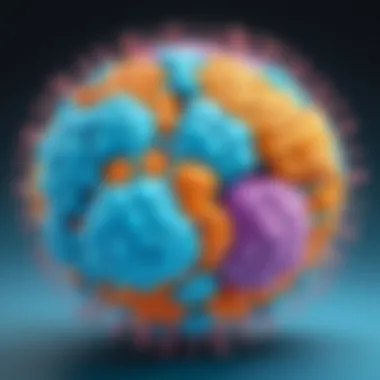
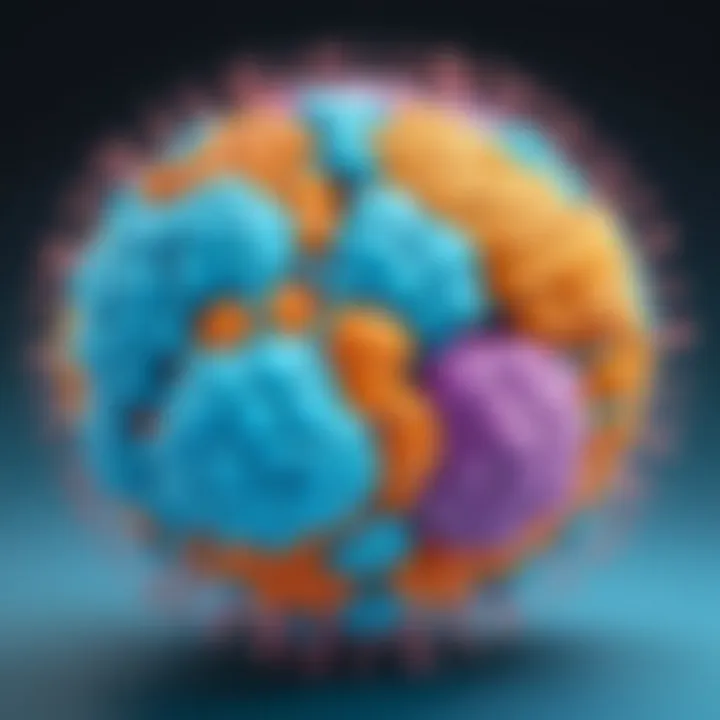
Intro
Translating RNA is a fundamental process in cellular biology, serving as a bridge between genetic information and functional proteins. This process occurs in all living organisms and is essential for life as we know it. In the following sections, we will explore the various components and mechanisms involved in RNA translation. We will analyze their roles and implications in genetics as well as biotechnology.
Understanding RNA translation is crucial, as it reveals the intricate workings of cells and how they adapt to environmental changes. The central role of messenger RNA (mRNA), transfer RNA (tRNA), and ribosomes in this process cannot be overstated. Each component performs a specific function, contributing to the overall efficiency and accuracy of protein synthesis.
"The significance of RNA translation extends beyond basic biology; it is at the heart of numerous biotechnological advancements."
Throughout this article, we will break down the stages of translation, delve into the finer points of each step, and examine how these mechanisms may pave the way for innovations in genetic engineering and therapeutics.
Overview of RNA Translation
The process of RNA translation is pivotal in the field of molecular biology. It is crucial because it bridges the gap between genetic information encoded in DNA and the functional proteins that execute various functions within a cell. Understanding translation mechanisms not only provides insight into how genes are expressed but also highlights the significance of proteins in sustaining life. In an era where biotechnology is revolutionizing medicine, agriculture, and industrial processes, a thorough comprehension of RNA translation is undeniably beneficial for researchers and practitioners.
In this article, we will explore the elaborate mechanisms of RNA translation. We will focus on key components involved such as messenger RNA (mRNA), transfer RNA (tRNA), and ribosomes. These elements collaborate seamlessly to create proteins by decoding the information carried by RNA. In addition, we will delve into the stages of translation, including initiation, elongation, and termination, providing a timeline perspective of how proteins are synthesized and modulated.
Definition and Importance
RNA translation can be defined as the biochemical process that converts messenger RNA sequences into a polypeptide chain of amino acids, ultimately folding into functional proteins. The importance of this process cannot be overstated—it defines how genetic code from DNA is manifest as tangible, active components within living organisms.
The roles of mRNA, tRNA, and rRNA are foundational. Messenger RNA acts as a template that carries the genetic information from the nucleus to ribosomes, where the translation occurs. Transfer RNA is responsible for bringing the appropriate amino acids to the growing polypeptide chain, ensuring accuracy in protein synthesis. Ribosomal RNA, as a structural component of ribosomes, creates the site for assembly. These interactions illustrate a fine-tuned orchestration that is vital for cellular functionality and adaptability.
The synthesis of proteins through RNA translation is not just a cellular necessity; it is a complex interplay of various molecules that sustain life.
Historical Context
The study of RNA translation has evolved significantly since its inception. Early discoveries in the 1950s and 1960s laid the groundwork for understanding how genetic information is translated into proteins. The elucidation of the genetic code by researchers like Marshall Nirenberg and Har Gobind Khorana marked a turning point. They demonstrated how sequences of nucleotides in mRNA correspond to specific amino acids.
As the years went by, advancements in molecular biology techniques revolutionized our comprehension of translation. Techniques such as genetic sequencing and later CRISPR technology have allowed scientists to manipulate and understand the complexity of RNA translation with great detail. Understanding historical milestones in this field highlights the cumulative effort of scientists who have contributed to the modern understanding of protein synthesis and genetic expression.
Types of RNA Involved in Translation
Understanding the types of RNA involved in translation is vital to grasp the complexities of protein synthesis. Each type of RNA plays a specific role, and together they facilitate the critical process of decoding the information stored in the genetic material. The importance of recognizing these types lies in their distinct functions:
- Messenger RNA (mRNA)
- Transfer RNA (tRNA)
- Ribosomal RNA (rRNA)
Messenger RNA (mRNA)
Messenger RNA is the template for protein synthesis. It carries the genetic blueprint from DNA in the nucleus to the ribosomes in the cytoplasm. The mRNA is synthesized during transcription, where RNA polymerase reads the DNA sequence and constructs a complementary RNA strand. This strand then undergoes processing, which includes splicing, capping, and polyadenylation, before it exits the nucleus.
The importance of mRNA cannot be overstated. It determines the sequence of amino acids in a protein, directly influencing its structure and function. Any change in the mRNA sequence can lead to significant changes in protein function, which can have various biological consequences. This illustrates how mRNA forms a direct link between genes and the resulting proteins that function within the cell.
Transfer RNA (tRNA)
Transfer RNA functions as the adapter molecule in protein synthesis. Each tRNA molecule carries a specific amino acid and has an anticodon that pairs with a corresponding codon on the mRNA strand during translation. This ensures that the correct amino acid is added to the growing polypeptide chain, a process that is crucial for producing functional proteins.
The efficiency of translation relies heavily on tRNA. There are different tRNA molecules for each amino acid, and indeed their availability and function affect protein synthesis rates. Moreover, defects in tRNA can lead to diseases, illustrating its significance in cellular function.
Ribosomal RNA (rRNA)
Ribosomal RNA is a fundamental component of ribosomes, the cellular machinery responsible for protein synthesis. The rRNA molecules help to form the ribosome structure, providing a scaffolding for the assembly of proteins and facilitating the interactions between mRNA and tRNA.
Ribosomes consist of two subunits, each composed of rRNA and protein. This complex work together to decode mRNA and catalyze the formation of peptide bonds between amino acids, which forms proteins. rRNA is central to the translation process's functionality and efficiency, making it indispensable for life.
The interplay between mRNA, tRNA, and rRNA is a critical element in the execution of translation, highlighting the collaborative nature of molecular biology.
Recognizing these types of RNA illuminates their contributions to translation. Such understanding enhances insights into genetic expression and the mechanisms underpinning various biological processes.
The Ribosome: Central Role in Translation
The ribosome is a fundamental component in the process of RNA translation, acting as the site where proteins are synthesized from messenger RNA. Its significance cannot be overstated. Ribosomes facilitate the decoding of mRNA, ensuring that the genetic information is accurately translated into a functional protein. This process is essential for maintaining the vitality of all living organisms, making ribosomes one of the most crucial molecular machines in cellular biology.
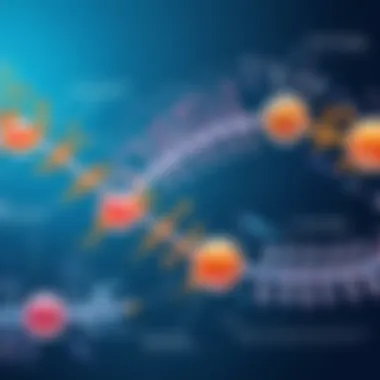
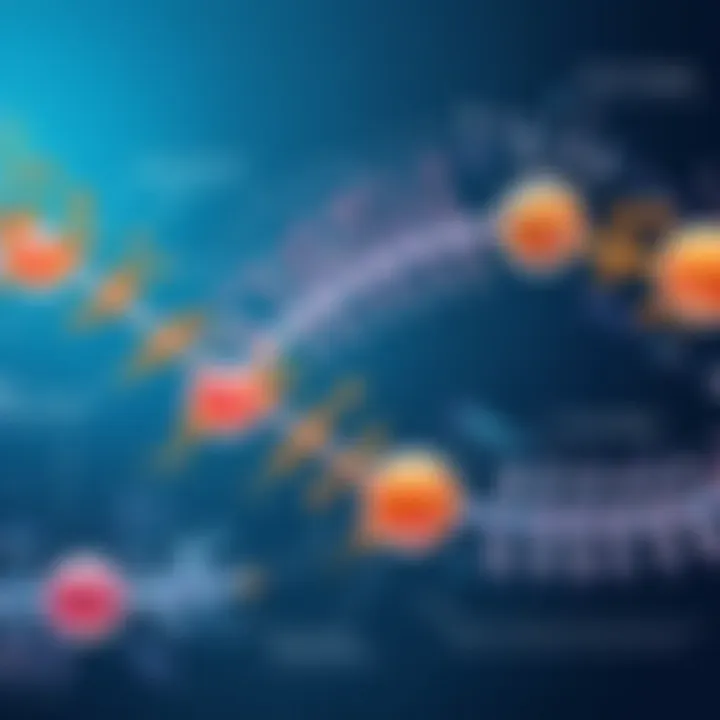
Ribosomes are composed of ribosomal RNA (rRNA) and proteins, forming a complex that enables them to perform their functions effectively. Understanding ribosomes also sheds light on various diseases linked to protein synthesis errors, which can arise from mutations in the ribosomal components or related factors. By exploring their structure and function, the implications of ribosomes in biotechnology and medicine can be better appreciated.
Structure of the Ribosome
The structure of the ribosome is intricate and highly organized. A ribosome is made up of two subunits: the large subunit and the small subunit. These subunits come together during the translation process.
- Small Subunit: The small subunit binds to the mRNA molecule. It is responsible for reading the sequence of codons, which are triplet nucleotides that correspond to specific amino acids.
- Large Subunit: The large subunit contains the peptidyl transferase center, where peptide bonds are formed between amino acids to create polypeptide chains.
Together, these subunits create a functional ribosome, ensuring that translation occurs efficiently. The binding sites in the ribosome include the A site (aminoacyl), P site (peptidyl), and E site (exit). This arrangement plays a critical role in the translation process by allowing tRNA molecules to sequentially deliver amino acids needed for protein synthesis.
Function of the Ribosome
The primary function of the ribosome is to facilitate the process of translation, wherein the genetic code carried by mRNA is converted into a specific polypeptide chain. This involves several key steps:
- Initiation: The ribosome assembles around the start codon of the mRNA with the first tRNA attached, setting the stage for translation.
- Elongation: As the ribosome moves along the mRNA strand, additional tRNA molecules bring successive amino acids to the growing polypeptide chain. This step relies on the ribosome's ability to accurately match tRNA anticodons to the corresponding mRNA codons.
- Termination: When a stop codon is encountered, the ribosome disassembles and releases the completed polypeptide chain, which will then fold into its functional protein structure.
"Ribosomes are not just passive machines; they are dynamic entities that adapt to their cellular environment, affecting the expression and regulation of genes."
The functionality of ribosomes is vital for cellular processes, as proteins produced are involved in nearly all aspects of life, from structure and signalling to enzyme activity. Their role extends into various fields, including drug development, where targeting the ribosomal function can lead to therapeutic advances. The ribosome's central role in translation exemplifies the intersection of molecular biology and applied sciences.
Stages of RNA Translation
Understanding the stages of RNA translation is crucial for grasping how genetic information is converted to functional proteins. The process unfolds in three main stages: initiation, elongation, and termination. Each stage plays a distinct role in ensuring that the translation process is precise and efficient. The interconnectivity of these stages highlights their importance in cellular function and the broader implications in biotechnology and medicine.
Initiation
Initiation marks the beginning of the translation process. During this phase, the small ribosomal subunit binds to the messenger RNA (mRNA) strand. This interaction occurs at the start codon, which is typically AUG. The initiation factors help in the assembly of the ribosome at this critical site.
Once the small subunit is bound to the mRNA, the transfer RNA (tRNA) carrying the appropriate amino acid pairs with the start codon. This connection is essential as it sets the reading frame for the entire translation process.
In eukaryotic cells, several additional factors, including the eukaryotic initiation factor 4E (eIF4E) and the poly(A)-binding protein, facilitate the recruitment of the large ribosomal subunit. This teamwork is essential for the successful transition into the next stage, elongation.
Elongation
Elongation follows initiation and is characterized by the sequential addition of amino acids to the growing polypeptide chain. With the large ribosomal subunit firmly attached, the ribosome has two important sites: the aminoacyl (A) site and the peptidyl (P) site.
In this stage, a new tRNA molecule enters the A site, bringing an amino acid that will be added to the chain. The ribosome facilitates the peptide bond formation between the amino acids in the P and A sites. As the polypeptide chain grows, the ribosome undergoes translocation, moving one codon down the mRNA strand, thus shifting the tRNA from the A site to the P site. This movement is a critical part of elongation, ensuring that the translation process continues smoothly.
As this stage continues, many ribosomes can translate a single mRNA strand simultaneously, allowing for a rapid increase in protein production. This efficiency showcases the ribosome's vital role in the cell, underpinning cellular functions and responses.
Termination
Termination concludes the process of translation. It occurs when the ribosome encounters a stop codon on the mRNA: UAA, UAG, or UGA. These codons do not correspond to any amino acid and signal the end of protein synthesis.
At this point, release factors bind to the A site, prompting the ribosome to catalyze the release of the completed polypeptide chain from the tRNA. This step is crucial as it allows for the newly synthesized protein to fold into its functional structure. The ribosome then disassembles and frees the mRNA for potential re-translation or decay.
The significance of the termination stage is evident in cellular regulation. Errors during this phase can lead to incomplete or dysfunctional proteins, which may contribute to diseases or disorders.
To summarize, the stages of RNA translation—initiation, elongation, and termination—are fundamental to protein synthesis, connecting the information encoded in mRNA to functional biochemical pathways.
Translation Regulation Mechanisms
Translation regulation mechanisms are crucial for organisms as they ensure that protein synthesis occurs precisely when needed. These mechanisms help maintain the balance of proteins in cells, adapting to various internal and external conditions. By regulating translation, cells can rapidly respond to changes in their environment or developmental stage. This control aids in optimizing energy usage and helps avoid the uncontrolled growth associated with diseases.
Translational Control in Cells
Translational control refers to how cells modulate the process of translation. It affects how effectively messenger RNA (mRNA) is translated into a protein. This regulation can occur at several points. Various mechanisms exist, such as the availability of initiation factors or the activation of specific mRNA sequences.
- mRNA availability: The quantity of mRNA available can directly impact translation efficiency. Cells can rapidly degrade unnecessary mRNA strands.
- Initiation factors: Proteins known as initiation factors play a role in the beginning of translation. Modulating these factors influences how quickly translation starts.
- MicroRNAs: These small regulatory RNA molecules can bind to mRNA and prevent protein synthesis. They serve as fine-tuners of gene expression, ensuring that proteins are not made in excess.
These controls are especially vital during stress or during changes in developmental stages. Understanding these mechanisms can provide insights into cellular behavior and adaptations, such as how tumor cells evade normal regulatory mechanisms.
Influence of External Factors
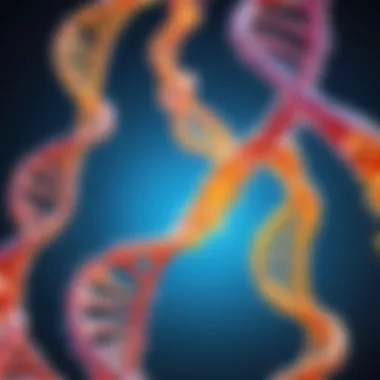
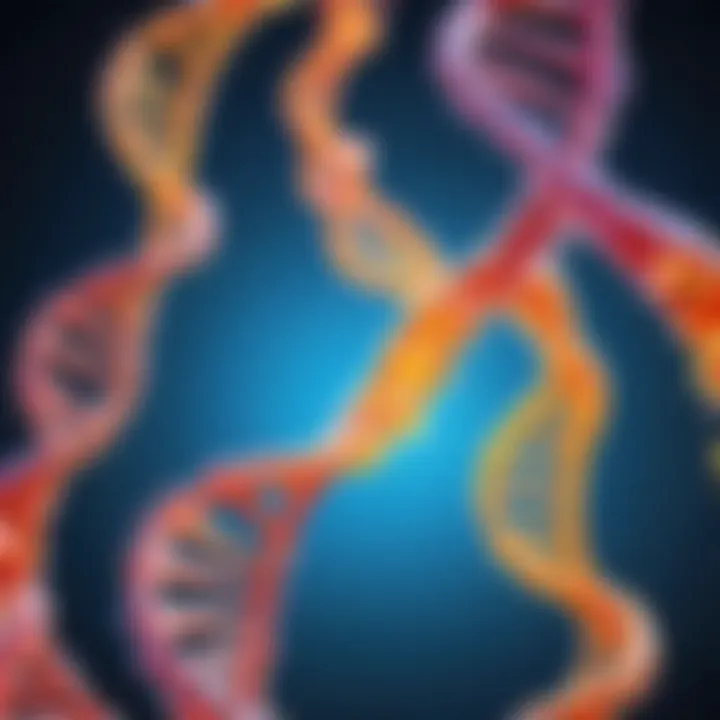
External factors can significantly influence translation regulation. These factors include nutrients, stress, and environmental conditions. When cells experience nutrient scarcity, they often slow down translation to conserve resources. Conversely, an abundance of specific nutrients can stimulate increased translation, particularly of growth-related proteins.
- Nutrient availability: The presence of amino acids plays a crucial role in signaling pathways that govern translation rates. Lack of amino acids leads to decreased protein synthesis.
- Stress signals: Cells under stress conditions, like hypoxia or heat shock, may adjust their translational machinery. This adjustment often results in prioritizing the synthesis of survival proteins while downregulating others.
- Hormonal regulation: Hormones such as insulin affect translation. For instance, insulin can activate pathways that promote protein synthesis in response to nutrient uptake.
"Translation regulation mechanisms allow cells to maintain homeostasis by adapting to their changing environments and ensuring efficient resource allocation."
The field of translation regulation is dynamic, as researchers unveil the complex interplay between these mechanisms and cellular processes. This understanding can lead to significant implications in medicine, especially for diseases resulting from dysregulated protein synthesis.
Mutations and Their Impact on Translation
Understanding mutations and their impact on translation is essential in the study of molecular biology and genetics. Mutations can influence how RNA is translated into proteins, which in turn can affect cellular functions and the integrity of biological systems. They play a critical role in evolution, disease, and biotechnology. This section will cover the various types of mutations, their significance, and their consequences on protein synthesis.
Types of Mutations
Mutations can take various forms, and they can arise from several factors such as environmental exposures or errors during DNA replication. Here are some common types:
- Substitution Mutations: One base is replaced by another. This may lead to a silent mutation (no change in protein), a missense mutation (a different amino acid), or a nonsense mutation (a premature stop codon).
- Insertion Mutations: Extra bases are added into the DNA sequence, which can disrupt subsequent amino acid coding.
- Deletion Mutations: Bases are removed from the sequence, often resulting in frameshift mutations, which alters the downstream protein structure.
Each mutation type can have different implications for the translation process, influencing how proteins are synthesized and functioning in the organism.
Consequences on Protein Synthesis
The impact of mutations on protein synthesis can be profound. The following implications illustrate this:
- Altered Protein Function: Missense mutations can lead to proteins that perform differently, potentially yielding nonfunctional or even harmful proteins.
- Disrupted Translation: Nonsense mutations can create stop codons too early in the mRNA sequence. This results in truncated proteins that cannot fulfill their biological roles.
- Frameshift Mutations: Both insertion and deletion mutations can shift the reading frame of the coding sequence, resulting in completely different amino acids being added, often leading to dysfunctional proteins.
"Mutations can be a source of new traits but can also lead to diseases, highlighting the delicate balance required in the translation process."
The consequences of these mutations extend beyond the individual protein. They can lead to significant changes in cellular behavior, potentially resulting in conditions such as cancer or genetic disorders. Thus, understanding the nature and impact of mutations on translation is crucial for advancements in medical research and therapeutic developments.
Applications of RNA Translation in Biotechnology
RNA translation has a profound impact on biotechnology. This area intersects with multiple scientific fields, allowing advancements in areas such as genetic engineering and therapeutic production. The ability to manipulate RNA and protein synthesis opens doors for new treatments and solutions in medicine.
Genetic Engineering Techniques
Genetic engineering utilizes RNA translation principles to modify organisms. This typically involves inserting specific genes into an organism's DNA. The goal is to produce proteins that can, for instance, enhance crop resilience or even create synthetic hormones. CRISPR-Cas9 technology is one such example where RNA guides the enzyme to specific DNA sequences for editing.
- Advantages:
- Precision in gene editing
- Ability to control traits in organisms
- Potential to eradicate genetic diseases
Challenges exist, including ethical considerations surrounding genetic modifications and the potential long-term effects on ecosystems. Therefore, ongoing research in this area is crucial to establish safe practices.
Therapeutic Protein Production
The aim of therapeutic protein production is to create proteins that can treat diseases. This often involves expressing human proteins in host cells, like Escherichia coli or yeast, through RNA translation. This process is key in producing insulin for diabetes treatment, monoclonal antibodies for cancers, and enzymes for various metabolic disorders.
- Key Outcomes:
- High yield of desired proteins
- Improved purity and functionality of products
- Faster response to emerging health crises
In summary, the applications of RNA translation in biotechnology not only facilitate numerous medical advances but also provoke relevant discussions on safety and ethics.
"The evolution of RNA-based technologies heralds a new era in personalized medicine and genetic research."
As these technologies develop, they elicit both enthusiasm and caution among scientists and ethicists alike.
Translation in Different Organisms
Understanding translation across different organisms is essential to grasp the diversity of life and the underlying mechanisms that drive biological processes. The difference between prokaryotic and eukaryotic translation illustrates evolutionary adaptations that enable organisms to thrive in various environments. Both processes possess unique characteristics that impact protein synthesis, efficiency, and regulation, ultimately relating to function and complexity in living organisms.
Prokaryotic Translation
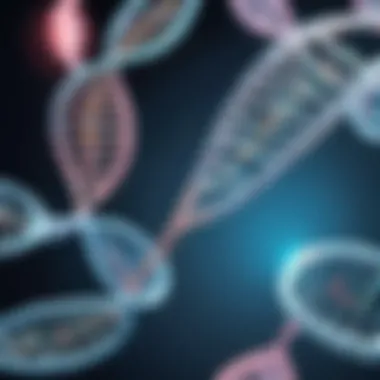
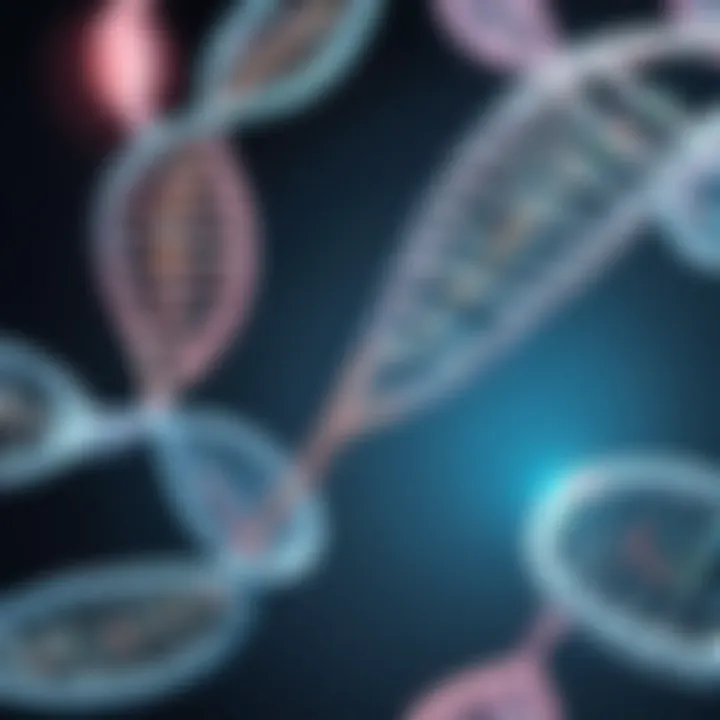
Prokaryotic translation is primarily characterized by its simplicity and speed. Prokaryotes, such as bacteria, lack a defined nucleus and organelles. Therefore, the processes of transcription and translation occur simultaneously in the cytoplasm. This coupling allows for rapid protein production, enabling bacteria to quickly respond to environmental changes.
Key features of prokaryotic translation include:
- Single ribosomal RNA structure: The ribosome consists of a smaller 30S subunit and a larger 50S subunit, which assemble at the mRNA.
- Initiation mechanisms: In prokaryotes, the ribosome identifies the start codon via a sequence called the Shine-Dalgarno sequence, which pairs with the ribosomal RNA for proper positioning.
- Polycistronic mRNA: Prokaryotic mRNAs often code for multiple proteins within a single strand, allowing for coordinated regulation of genes that are functionally related.
Despite their simplicity, prokaryotic mechanisms underline complex machinery that sustains life, contributing to processes such as nutrient cycling and disease propagation. Insights from prokaryotic translation inform areas in biotechnology and synthetic biology, enhancing our understanding of fundamental biological concepts.
Eukaryotic Translation
Eukaryotic translation is markedly more complex, involving various cellular compartments and diverse regulatory mechanisms. Eukaryotic cells differentiate into specialized types, with a defined nucleus containing DNA. This structural separation creates layered regulatory pathways that dictate how and when proteins are synthesized.
Key distinctions in eukaryotic translation include:
- Nuclear processing: Before translation, eukaryotic mRNA undergoes capping, polyadenylation, and splicing. This modification process is crucial for stability and export from the nucleus to the cytoplasm where translation occurs.
- Ribosome composition: Eukaryotic ribosomes are larger than their prokaryotic counterparts, consisting of a 40S small subunit and a 60S large subunit. This complexity allows for intricate protein synthesis.
- Monocistronic mRNA: Most eukaryotic mRNAs code for a single protein, providing an additional level of regulation for gene expression compared to prokaryotes.
Eukaryotic translation reflects the sophistication of multicellular life, enabling diverse cellular functions essential for development and specialization in organisms. Understanding these systems can lead to innovations in therapeutics and advancements in genetic research.
Technological Advances in RNA Translation Research
The growth of scientific knowledge in RNA translation has been greatly enhanced by technological advances. These developments not only provide insights into the fundamental processes of life but also open new avenues for research and applications. Understanding how these technologies impact RNA translation can lead to significant breakthroughs in fields such as biotechnology and medicine.
Next-Generation Sequencing
Next-generation sequencing, commonly known as NGS, has revolutionized how scientists study RNA. This technology allows for high-throughput sequencing of RNA samples, enabling researchers to identify the entire transcriptome of an organism simultaneously. NGS can detect low-abundance transcripts and alternative splicing events that were previously challenging to analyze.
The benefits of NGS in RNA research are numerous:
- High Throughput: This technology can sequence millions of fragments of RNA at once, reducing time and costs associated with conventional methods.
- Depth of Coverage: NGS offers deeper coverage of samples, allowing for more accurate representation of gene expression levels.
- Diverse Applications: It can be applied in various contexts, such as discovering novel RNA species, studying mutations, and profiling cellular responses to different stimuli.
NGS has significant implications for understanding diseases at the molecular level. For instance, identifying RNA changes in cancer cells can lead to targeted therapies that specifically address the alterations found in the RNA. Moreover, combining NGS with other technologies can help researchers gain a better understanding of regulatory mechanisms governing RNA translation.
CRISPR Technology
CRISPR technology has emerged as a powerful tool for gene editing and manipulation. Originally discovered as part of the bacterial immune system, CRISPR has been adapted to target specific RNA sequences for various applications in RNA translation.
CRISPR’s implications in RNA translation are profound:
- Gene Editing: Researchers can use CRISPR to edit RNA directly, allowing for precision in modifying genes that influence translation.
- Regulatory Elements: CRISPR can facilitate the study of RNA regulatory elements by enabling targeted modifications and observing the effects on translation efficiency.
- Therapeutic Applications: The ability to control RNA translation can lead to novel therapies for genetic disorders.
CRISPR technology, therefore, plays a crucial role in advancing our understanding of RNA translation mechanisms. By enabling precise manipulation of RNA, it opens doors to innovative therapeutic strategies.
"The integration of technological advances such as NGS and CRISPR in RNA research enhances our ability to comprehend and manipulate the cellular processes that impact life itself."
Future Directions in RNA Translation Studies
As research in RNA translation progresses, several intriguing frontiers are emerging. These directions hold significant promise for enhancing our understanding of molecular biology and expanding the therapeutic landscape. Understanding these future areas is important, as they can lead to breakthroughs in genetic research and disease treatment.
Emerging Research Areas
Recent advancements in biotechnology and molecular genetics are paving the way for novel investigative fields. Topics like ribosome profiling and RNA structure determination are gaining traction. Ribosome profiling enables scientists to observe how ribosomes translate mRNA in real-time. This technique can yield insights into the dynamics of translation, like how different conditions affect protein synthesis. Conducting research in RNA secondary and tertiary structures can further unveil how RNA's shape affects its function in translation.
Another compelling area is the exploration of non-coding RNAs. These RNA types, which do not code for proteins, have crucial roles in regulating gene expression. Identifying their mechanisms can redefine our understanding of translation and gene control.
Some key areas for investigation include:
- Ribosome profiling techniques
- Non-coding RNA functionality
- RNA interactomics
Potential for Novel Therapies
The implications of advancing RNA translation studies transcend theoretical research. They provide the groundwork for innovative therapeutic approaches. For instance, targeted therapies often utilize RNA molecules. By understanding translation intricacies, researchers can design RNA-based drugs or interventions that can effectively target specific diseases.
CRISPR technology is also evolving, with more precise methods for editing RNA sequences. This can change how genetic disorders are treated. Instead of altering DNA directly, which has downstream effects, editing the RNA could offer a more contained and manageable solution. The goal is to develop therapies that directly correct the faulty RNA process leading to disease.
"Advancing our understanding of RNA translation can profoundly change how we approach genetic diseases and therapies."
Moreover, the exploration of mRNA as a therapeutic tool, akin to the COVID-19 vaccines, is just the beginning. Continued research may lead to more vaccines or treatments for other diseases, substantially impacting global health.
In summary, future directions in RNA translation studies are rich with potential. They promise novel therapies that can help address unanswered medical questions and enable better understanding of disease mechanisms. This ongoing frontier of science challenges researchers to think critically and innovatively in addressing some of the world's toughest health hurdles.